The Power of Mirror Symmetry
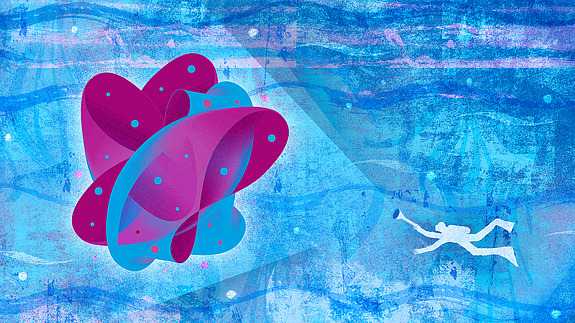
Ideas that originate in particle physics have an uncanny tendency to appear in the most diverse mathematical fields. This is especially true for string theory. Its stimulating influence in mathematics will have a lasting and rewarding impact, whatever its final role in fundamental physics turns out to be. The number of disciplines that it touches is dizzying: analysis, geometry, algebra, topology, representation theory, combinatorics, probability—the list goes on and on.
A striking example of the magic of quantum theory is mirror symmetry— a truly astonishing equivalence of spaces that has revolutionized geometry. The story starts in enumerative geometry, a well-established but not very exciting branch of algebraic geometry that counts objects. For example, researchers might want to count the number of curves on Calabi-Yau spaces—six-dimensional solutions of Einstein’s equations of gravity that are of particular interest in string theory, where they are used to curl up extra space dimensions.
Just as you can wrap a rubber band around a cylinder multiple times, the curves on a Calabi-Yau space are classified by an integer, called the degree, that measures how often they wrap around. Finding the numbers of curves of a given degree is a famously hard problem, even for the simplest Calabi-Yau space, the so-called quintic. A classical result from the nineteenth century states that the number of lines— degree-one curves—is equal to 2,875. The number of degree-two curves was only computed around 1980 and turns out to be much larger: 609,250. But the number of curves of degree three required the help of string theorists.
Around 1990, a group of string theorists asked geometers to calculate this number. The geometers devised a complicated computer program and came back with an answer. But the string theorists suspected it was erroneous, which suggested a mistake in the code. Upon checking, the geometers confirmed there was, but how did the physicists know?
String theorists had already been working to translate this geometric problem into a physical one. In doing so, they had developed a way to calculate the number of curves of any degree all at once. It’s hard to overestimate the shock of this result in mathematical circles. It was a bit like devising a way to climb each and every mountain, no matter how high!
Within quantum theory it makes perfect sense to combine the numbers of curves of all degrees into a single elegant function. Assembled in this way, it has a straightforward physical interpretation. It can be seen as a probability amplitude for a string propagating in the Calabi–Yau space, where the sum-over-histories principle has been applied. A string can be thought to probe all possible curves of every possible degree at the same time and is thus a super-efficient “quantum calculator.”
But a second ingredient was necessary to find the actual solution: an equivalent formulation of the physics using a so-called “mirror” Calabi–Yau space. The term “mirror” is deceptively simple. In contrast to the way an ordinary mirror reflects an image, here the original space and its mirror are of very different shapes; they do not even have the same topology. But in the realm of quantum theory, they share many properties. In particular, the string propagation in both spaces turns out to be identical. The difficult computation on the original manifold translates into a much simpler expression on the mirror manifold, where it can be computed by a single integral. Et voilà!
Mirror symmetry illustrates a powerful property of quantum theory called duality: Two classical models can become equivalent when considered as quantum systems, as if a magic wand is waved and all the differences suddenly disappear. Dualities point to deep but often mysterious symmetries of the underlying quantum theory. In general, they are poorly understood and an indication that our understanding of quantum theory is incomplete at best.
The first and most famous example of such an equivalence is the well-known particle-wave duality that states that every quantum particle, such as an electron, can be considered both as a particle and as a wave. Both points of views have their advantages, offering different perspectives on the same physical phenomenon. The “correct” point of view—particle or wave—is determined solely by the nature of the question, not by the nature of the electron. The two sides of mirror symmetry offer dual and equally valid perspectives on “quantum geometry.”
Mathematics has the wonderful ability to connect different worlds. The most overlooked symbol in any equation is the humble equal sign. Mirror symmetry is a perfect example of the power of the equal sign. It is capable of connecting two different mathematical worlds. One is the realm of symplectic geometry, the branch of mathematics that underlies much of mechanics. On the other side is the realm of algebraic geometry, the world of complex numbers. Quantum physics allows ideas to flow freely from one field to the other and provides an unexpected “grand unification” of these two mathematical disciplines.
It is comforting to see how mathematics has been able to absorb so much of the intuitive, often imprecise reasoning of quantum physics and string theory, and to transform many of these ideas into rigorous statements and proofs. Mathematicians are close to applying this exactitude to homological mirror symmetry, a program that vastly extends string theory’s original idea of mirror symmetry. In a sense, they’re writing a full dictionary of the objects that appear in the two separate mathematical worlds, including all the relations they satisfy. Remarkably, these proofs often do not follow the path that physical arguments had suggested. It is apparently not the role of mathematicians to clean up after physicists! On the contrary, in many cases completely new lines of thought had to be developed in order to find the proofs. This is further evidence of the deep and as yet undiscovered logic that underlies quantum theory and, ultimately, reality.